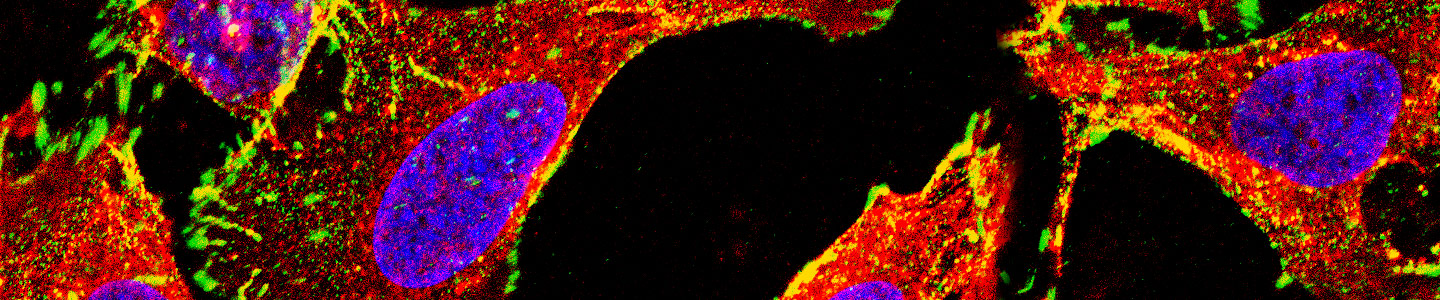
Authors: Elizabeth Turner Gillies, PhD, and Fang Tian, PhD
The shift towards targeted cancer therapies, which began with the development of tamoxifen and accelerated following the success of drugs like rituximab and imatinib (Gleevec), has changed the way we think about cancer treatment and prognosis. By targeting the specific molecular pathways driving tumor proliferation rather than killing all rapidly dividing cells as in traditional chemotherapy, targeted therapies have the potential to turn a dire prognosis into a manageable condition.1 However, despite encouraging progress in this area, low clinical trial success rates, difficulties with cancer biomarker identification and testing, and the rapid emergence of drug resistance present significant challenges toward developing the next generation of targeted cancer therapies.2
The discovery and development of new therapies for current and emerging cancer targets relies heavily on the high-throughput screening and iterative refinement of lead compounds and biologics.3 The currently available cancer model systems for targeted drug screening are typically derived from either commonly used tumor cell lines or from established cell lines that have been modified to overexpress specific drug targets. Both model types have their respective pros and cons. Primary or spontaneously immortalized tumor cells more closely model the genetic and phenotypic heterogeneity found in clinical tumor samples, but this heterogeneity is rarely fully characterized or defined, so results can be difficult to interpret. Even when well-characterized cancer lines are used as screening models, screening of therapies targeting a specific oncogenic mechanism is confounded by the complex web of mechanisms driving tumor growth. In contrast, models derived from cell lines modified to overexpress a particular cancer biomarker have a clear oncogenic mechanism. However, expression of an oncogene at artificially high, non-physiological levels in a cell that would otherwise be non-cancerous doesn’t adequately model the complex cascade of events that would result in the formation of a tumor in vivo. These tradeoffs are significant contributors to the low clinical trial success rates for new targeted cancer therapies.
To address this issue, ATCC has been steadily expanding an array of CRISPR/Cas9 genome-editing capabilities and leveraging its extensive library of human cell lines for the development of custom-engineered, biomarker-specific human cancer models. By using CRISPR-based genome engineering to make precise changes to the genome of a target cell line, we can now alter those lines in a way that closely mimics the spontaneous development of cancer in a healthy tissue or specific cancer genotypes found in clinical patient samples.4 For example, ATCC has developed a lung adenocarcinoma model with an engineered EML4/ALK oncogenic fusion that functions in the same way as spontaneous EML4/ALK rearrangements isolated from clinical tumor samples.5 This new cell line is an ideal model system for developing and screening new ALK inhibitor therapies. ATCC has also used CRISPR/Cas9 to create a model glioma line with an engineered IDH1 R132H allele to recapitulate the way cellular metabolism is altered in IDH1 mutant gliomas.10 Because the R132H mutation was engineered directly into the endogenous IDH1 gene locus of this model line, rather than overexpressed from a third IDH1 gene inserted elsewhere in the genome, the model shows levels of D-2-hydroxygluterate accumulation in line with clinical IDH1 mutant glioma samples. This added degree of physiological relevance makes the new glioma model both a useful tool for IDH1 targeted drug screening and an accurate IDH1 biomarker reference model. Similarly, an engineered IDH2 R140Q mutant cell model for acute myeloid leukemia was developed that mimics the genetic alternation and metabolic changes that drive progression of AML.6 Again, this engineered cancer model shows physiologically relevant levels of 2-D-hydroxygluterate accumulation and responds to IDH2 targeted inhibitors in the same way as clinical AML isolates. Since these modified cell lines are cultured in a manner virtually identical to their parental lines, these new gene-edited cancer models are an easy substitute for historical cancer lines or overexpression model for drug discovery and development. Furthermore, unlike traditional cancer model systems, gene-edited cancer models come with a built-in isogenic control with an identical genetic background, which allows for early identification of biomarker-specific drug responses. Because gene-edited cancer models are both biologically relevant and biomarker-specific, they have the potential to dramatically increase clinical trial success rates and accelerate the development of targeted cancer therapeutics.
Resources for your CRISPR/Cas9-engineered cancer research
EMT Reporter Models for Cancer Research: A Window into Invasion and Metastasis
ATCC used CRISPR to create reporter cells that track epithelial-mesenchymal transition in real time. Watch the webinar to learn how we did it.
Clinical outcomes in targeted cancer therapy are often compromised by the development of resistance to targeted therapies in patients who receive these treatments. This acquired resistance to targeted therapies in cancer is reminiscent of the rise of antibiotic resistance in bacterial infections; patients who initially respond well to a targeted cancer therapy later develop tumors that are resistant to that type of therapy. The most notable example of this phenomenon is the development of resistance to BRAF inhibitor therapy in melanoma patients.7 Initially, BRAF inhibitors like dabrafenib and vemurafenib are highly effective for the treatment of metastatic melanomas with a BRAF V600E mutation.8 Tumors shrink and disappear but can reappear 6 months later, now resistant to BRAF inhibitors. There is an urgent need for new cancer therapies and combination treatment regimens to treat these resistant tumors. Screening of these new therapies relies on drug-resistant cancer models that carry the oncogenes that resulted in the initial onset of cancer as well as additional mutations that render them resistant to targeted therapy. Most traditional cancer models do not accurately represent this complexity, posing a significant barrier to the development of cancer therapies designed specifically to address treatment-resistant tumors.
ATCC offers a series of genome-edited melanoma model cell lines designed specifically to meet the demand for improved drug-resistant cancer models. Using CRISPR/Cas9, we engineered a series of BRAF V600E melanoma model lines that express point mutations associated with acquired BRAF inhibitor resistance into genes acting either upstream or downstream of BRAF in the RAS/Raf/MEK/ERK kinase signaling pathway.9-10 Two of these point mutations, NRAS Q61K and KRAS G13D, confer levels of resistance to the BRAF inhibitor that is consistent with the resistance seen in clinical tumor samples. Because these engineered NRAS and KRAS mutant melanoma lines can be paired with the parental melanoma cells they were derived from, they are a more advanced and biologically relevant cancer model system for screening new targeted therapies. ATCC has also developed another CRISPR/Cas9-engineered BRAF V600E melanoma model line that carries a Q56P mutation in MEK1 that renders these cells resistant to both BRAF and MEK targeted inhibitors. This isogenic melanoma model line is more sensitive to the commonly used BRAF/MEK inhibitor combination than to any equivalent dose of either inhibitor alone, highlighting the utility of this line for the development of combination therapies targeting tumors with acquired-inhibitor resistance. These types of custom-engineered cancer models are essential tools for the development of the next generation of targeted cancer therapies.
Although progress in the field of targeted cancer therapies can at times seem frustratingly slow, the development of improved cancer cell models is giving a much-needed boost to the targeted-therapy pipeline. With CRISPR/Cas9 genome-editing technology, we can now engineer cancer models that mimic specific cancer genotypes and phenotypes found in clinical tumor samples. Because these new cancer models are both biologically relevant and biomarker specific, they can greatly enhance the ability of drug developers to identify new compounds and biologics that will perform well in clinical trials. Furthermore, the development of systems that model acquired-inhibitor resistance is essential given the rise of drug-resistant tumors following treatment with certain targeted cancer therapies. CRISPR/Cas9 is the perfect tool for the precision engineering of cell models that mimic the genotype and phenotype of acquired inhibitor resistance. With these custom-engineered cell models, we can look forward to exciting new developments in targeted cancer therapy.
Download a PDF of this white paper
Download NowReferences
- Aggarwal S. Targeted cancer therapies. Nat Rev Drug Discov. 9(6): 427-428, 2010.
- Huang M, et al. Molecularly targeted cancer therapy: some lessons from the past decade. Trends Pharmacol Sci. 35(1): 41-50, 2014.
- Suggitt M, Bibby MC. 50 years of preclinical anticancer drug screening: empirical to target-driven approaches. Clin Cancer Res. 11(3): 971-981, 2005.
- Vogelstein B, et al. Cancer genome landscapes. Science. 339(6127): 1546-1558, 2013.
- Sasaki T, et al. The biology and treatment of EML4-ALK non-small cell lung cancer. Eur J Cancer. 46(10): 1773-1780, 2010.
- Mondesir J, et al. IDH1 and IDH2 mutations as novel therapeutic targets: current perspectives. J Blood Med. 2016; 7: 171–180, 2016.
- Davies H, et al. Mutations of the BRAF gene in human cancer. Nature. 417(6892): 949-954, 2002.
- Chapman PB, et al. Improved survival with vemurafenib in melanoma with BRAF V600E mutation. N Engl J Med. 364(26): 2507-2516, 2011.
- Nazarian R, et al. Melanomas acquire resistance to B-RAF(V600E) inhibition by RTK or N-RAS upregulation. Nature. 468(7326): 973-977, 2010.
- Holderfield M, et al. Targeting RAF kinases for cancer therapy: BRAF-mutated melanoma and beyond. Nat Rev Cancer. 14(7): 455-467, 2014.